Sie sind hier: Landwirtschaft > Transgene Pflanzen > Genet. Pflanzenschutz > Genet. erzeugte Resistenz Dieser Artikel ist leider nicht in deutscher Sprache verfügbar. 
3 State of the Art Genetic Engineering for Plant Protection
3.1 Resistance of Transgenic Plants
Strategies using genetic engineering to achieve disease and pest resistant
plants are advancing rapidly. Different degrees of resistance against
insects, viruses, fungi and bacteria have been reached with various
crop species. Table 3.1 summarizes the principal approaches and the
state of development. The following chapters describe these approaches
in more detail. Safety aspects of these approaches are discussed in
chapter 4.1.
3.1.1 Resistance to Plant-Feeding Insects
World-wide losses resulting from plant-feeding insects are still more than
10 % of total production, despite the intensive use of a large number
of chemical insecticides.[7] The use of these agrochemicals
is not only an important economical factor in agriculture, with annual
expenses of more than $7.8 billion world-wide, but has also often
resulted in ecological problems.[1] In addition, more than
500 target insect species have developed resistance to chemical
insecticides.[8] For these reasons, several approaches of
genetically engineered insect resistance have been developed. The use
of Bacillus thuringiensis toxins has by far been the most widely used
and successful strategy, and several Bt expessing crops have obtained
marketing clearance (see chapter 4.4). Some other approaches for insect
resistance in transgenic plants are in development and may lead to
successful insect resistance in the near future.
3.1.1.1 Bacillus Thuringiensis δ-Endotoxins
Biological Basis
Bacillus thuringiensis (Bt) is a common soil bacterium which forms resistant
resting structures under adverse conditions, so called spores. During
formation of these spores, Bt produces a crystal which is predominantly
comprised of one or more proteins called δ-endotoxins, Bt
toxins or insecticidal crystal proteins. These δ-endotoxins
possess insecticidal activity when ingested by certain insects.
Intensive investigations have led to detailed knowledge of the
mechanism and specificity of Bt toxin activity. Upon ingestion by
susceptible insect larvae, the crystalline inclusions are solubilized
in the midgut, releasing one or more proteins of different sizes.
Rapid proteolytic cleavage of these polypeptides then produces the
active toxic proteins. These activated toxins bind to specific
high-affinity receptors on the insect midgut cell membranes, leading
to the formation of pores that disturb the cellular osmotic balance.
Within minutes, midgut cells are paralyzed and disrupted.
[9]
Bacillus thuringiensis bacteria have been used since 1938 to produce an
insecticidal spray against certain insect pests. These products were,
and still are, produced by fermentation of single Bt strains in crude,
inexpensive media. Numerous strains of Bt are currently known. Each
strain produces differing numbers of δ-endotoxins with various
insecticidal activities. A given δ-endotoxin is typically
insecticidal only towards a narrow spectrum of insect targets. Screening
efforts have yielded strains insecticidal to Lepidoptera, Diptera,
Coleoptera, and even some strains effective against nematodes. Although
Bt bacteria are an effective microbial pesticide, a number of biological
constraints limit their use: The Bt δ-endotoxins are short lived
when sprayed on crops, thereby reducing the residual activity and
necessitating many applications during a growing season. Bt, as with
other spray-on insecticides, is difficult to deliver to insect species
which burrow into their host plant, hide under leaves or live primarily
under the soil surface[8]
Purpose |
Introduced Gene Coding for |
Source of Gene |
Mode of Action |
State of Development |
Insect resistance |
Modified Bacillus thuringiensis-δ-endotoxin |
Bacillus thuringiensis (Bacterium) |
Protein toxic for certain insect species |
More than 100 field trials with more than 10 different species,
including elite cultivars of important crops, permit for commercial
introduction of transgenic maize, potato and cotton in th USA |
Protease inhibitors, amylase inhibitors |
Plants |
Inhibition protein- or starchdegrading enzymes of plantfeeding
insects |
Laboratory/greenhouse experiments, some field trials with model
plants (tobacco), potato and rapeseed |
Lecitins (e.g. wheat germ agglutinin) |
Plants |
Protein toxic if ingested, mechanism unkown, probably midgut cell
disruption or nutrient absorption inhibition |
Field trials with model plants and maize |
Virus resistance |
Viral coat protein |
Pathogenic virus |
Unclear, interference with viral replication. Effect similar to
disease attenuation with non-pathogenic viruses. |
More than 100 field trials with more than 20 different species,
including elite cultivars of important crops. Commercialisation
permit of first varieties in the USA expeted in 1995. |
Replicase |
Pathogenic virus |
See coat protein-mediated protection |
Laboratory/greenhouse experiments with model plants |
Antisense mRNA of viral coat protein |
Pathogenic virus |
Complementation with viral RNA |
Laboratory/greenhouse experiments with model plants, field trials
with tobacco and melon |
mRNA of Satellite RNA |
Satellite RNA of pathogenic virus |
See coat protein-mediated protection |
Laboratory/greenhouse experiments, field trials in China |
mRNA of defective viral RNA |
Pathogenic virus |
See coat protein-mediated protection |
Laboratory/greenhouse experiments with model plants |
Ribozyme |
Various |
Cleavage of pathogen-mRNA |
Laboratory experiments |
Fungal resistance |
Chitinase, glucanase |
Plants, bacteria |
Breakdown of chitin or glucan in cell wall of fungi |
Field trials with several crops |
Ribosome inactivating proteins (RIPs) |
Plants |
Cleavage of foreign ribosomal RNA |
Field trial with model plants |
Stilbene synthase |
Plants |
Production of a phytoalexin, a natural antimicrobical agent |
Field trials with model plants |
Osmotin |
Plants |
Increased production of a natural anitmicrobical agent |
Field trials with several crops |
Pathogenesis related genes, e.g. PR-1a |
Plants |
Higher expression of genes which are involved in natural plant
response to diseases may lead to improved disease resistance |
Field trials with several crops |
RNAse |
Bacteria (Bacillus amyloliquefaciens) |
Artificial generation of hypersensitive cell death in infected plant
cells |
Laboratory/greenhouse experiments with model plants |
Bacterial resistance |
Cecropin |
Hyalophora cecropia (silkworm, insect) |
Damage of inner membrane of bacteria |
Field trials (e.g. potato) |
Lysozyme |
Various (chicken, bacteriophage) |
Destruction of bacterial cell wall murein |
Field trials (e.g. potato) |
Thionin |
Plants |
Antimicrobical activity, mechanism unknown |
Laboratory/greenhouse experiments with model plants |
Toxin-detoxifying enzymes |
Bacteria |
Detoxification of bacterial toxin which causes disease |
Laboratory/greenhouse experiments with model plants |
Toxin-resistant enzymes |
Bacteria |
Plant enzyme susceptible ot toxin replaced by toxin-resistant enzyme |
Laboratory/greenhouse experiments with model plants |
Table 3.1: Strategies used to archieve pest or disease resistant transgenic
plants (see text for sources).
These limitations can be overcome by expressing Bt toxins in transgenic
plants. Since Bt toxin is the product of a single gene, and its safe
use and efficacy has a long history, the δ-endotoxin genes were
among the first genes of commercial interest to be engineered into
plants. In the beginning, plants were transformed with full length Bt
toxin genes. These plants mostly showed only low levels of toxin mRNA
and protein and did not exert significant insecticidal activity. Better
results were obtained using truncated versions of Bt genes, but in
many cases the level of Bt toxin expression and therefore protection
was still to low to be of agronomic relevance.[8]
Significant increases were achieved by modification of the truncated
structural gene that had either none or only a minor effect on the
encoded amino acid sequence.[9] Alterations affected 5'-or 3'-
terminal regulatory mRNA sequences, mRNA secondary structure, G+C content
and/or codon usage. For example, Koziel et al. (1993) synthesized a
truncated crylA(b) gene encoding amino acids 1-648 of crylA(b) gene
from Bacillus thuringiensis var. kurstaki HD-1. This synthetic gene was
completely redesigned to replace the bacterial codons with maize-
preferred codons. The synthetic gene was combined with different
promoters and brought into maize. Field trials showed that the transgenic
maize lines provided season-long protection from repeated heavy
infestations of European corn borer (Ostrinia nubilalis, Lepidoptera),
which totally devastated control plants.[10]
Although the redesign of the δ-endotoxin genes is a successful means
to enhance the Bt toxin production in plants, it suffers from the
disadvantage of being laborious and expensive. In a recently published
paper, a promising new solution for enhanced translation efficiency of
Bt toxin genes was reported.[11] In this work, the transgene
was not introduced into the nucleus, but into plastids, a group of
small organelles of plant cells including chloroplasts with an own
genome called the plastone. The plastids are thought to be derived
from endosymbiontic bacteria and therefore have a transcriptional and
translational machinery of procaryotic origin. An unmodified Bt toxin
gene was incorporated into the tobacco plastid genome by plastid
transformation. Since a plant cell may contain up to 50'000 copies of
a plastid, the δ-endotoxin gene was present in several thousands
of copies per cell in transgenic plants. The plastid transformation
resulted in an accumulation of an unprecedented 3-5 % of soluble
protein of tobacco cells as Bt toxin and an extremely high toxicity
to the insects Heliothis virescens, Helicoverpa zea and Spodoptera
exigua. Apart from the high expression level of unaltered Bt genes,
the new method of plastid transformation has the additional advantages
that position effects can be avoided because the site of introduction
of the transgene can be targeted and the transgenes contained in the
plastids are not transmitted to other plants by pollen.[11]
State of Development
As already mentioned, transgenic maize, potato and cotton expressing Bt
toxins are among the first transgenic plants arriving on the market in
the USA. During the last years, hundreds of field trials in several
countries have proven the efficacy of this approach in controlling
important insect pests. Table 3.2 indicates field-tested crops
expressing Bt toxins. At present, the majority of transgenic crops
containing Bt genes have been transformed with cryI genes which have
activity against the lepidopterans, including the tobacco hornworm,
tobacco budworm, tomato pinworm, corn earworm and European corn borer.
More recently, the cryIIIA gene has been engineered into plants for
protection against coleopterans, including the Colorado potato beetle.
Bt δ-endotoxin genes with activity against insects of the genus
Diabrotica, such as corn rootworm, a major maize pest, will likely be
produced in the next generation of transgenic plants.[8]
Crop |
Number of Companies / Public Institutions involved |
Crop |
Number of Companies / Public Institutions involved |
Alfalfa |
1 / - |
Poplar |
- / 1 |
Apple |
- / 1 |
Potato |
4 / 2 |
Cotton |
6 / - |
Rice |
- / 1 |
Cranberry |
- / 1 |
Spruce |
- / 1 |
Eggplant |
- / 1 |
Tobacco |
4 / 1 |
Maize |
13 / 1 |
Tomato |
5 / - |
Oilseed rape |
1 / 2 |
Walnut |
- / 1 |
Table 3.2: Field tested crops expressing Bt toxins in the European Union and
the USA. Compiled from [12] and [13]
3.1.1.2 Inhibition of Insect Digestive Enzymes
Biological Basis
Many plant species protect themselves against plant-feeding insects by
producing proteins which interfere with insect digestion. Proteases
for example are enzymes which brake down proteins in the insect
digestive system. Protease inhibitors block the action of these
proteases. Most plant protease inhibitors do not effect endogenous
plant proteases, but specifically inhibit animal and microbial protease
enzymes. This suggests they may be involved in the protection of
vulnerable plant tissues from pest and pathogen attack by antinutritional
interaction with digestive enzymes. Protease inhibitors are widely
distributed within the plant kingdom and can accumulate to
particularly high levels in seeds and storage organs. The detrimental
effect of protease inhibitors was proven by feeding insects an
artificial diet. Rapid systemic accumulation of protease inhibitor
mRNA and _proteins was demonstrated in tomato and potato in response
to insect attack or mechanical wounding.[9] Another enzyme
interfering with insect digestion is α-amylase inhibitor
(αAI). This inhibitor blocks the action of α-amylase, an
enzyme used by insects to degrade starch.[14]
State of Development
The first protease inhibitor protein expressed in transgenic plants was the
cowpea trypsin inhibitor (CpTI). A full length CpTI DNA sequence was
brought under control of the CaMV 35S promoter and transferred into
tobacco. Bioassays considering insect biomass and survival and
percentage of leaf area have conclusively established that
CpTI-expressing plants have significant resistance to Heliothis
virescens and to a broad range of lepidopteran pests feeding on tobacco.
Recent field trials with transgenic tobacco expressing CpTI demonstrated
that CpTI had a negative effect on both larval survival and plant damage,
but the effect was not always significant. The tomato protease inhibitors
PH and the potato proteinase inhibitor PPI II represent two other
proteinase inhibitors which have been introduced into plants. They
adversely affected some insect species like Manduca sexta (tobacco
hornworm, lepidoptera) and Chrysodeixis eriosoma (green looper,
lepidoptera). In contrast, no inhibition of feeding was achieved with
transgenic tobacco producing PI-I inhibitor from tomato or soybean
Kunitz trypsin inhibitor (SBTI), indicating that not every protease
inhibitor can be employed for control of a specific pest species.
[15)
Two recent developments made possible the breeding of peas resistant to
bruchid beetles, important pests of seeds in post harvest storage,
by using α-amylase inhibitor ( αAI). First, it was shown
that the growth of two bruchid species is inhibited by low doses of
bean αAI, and second, a system for genetic transformation of
garden pea became available. The coding sequence of the bean
αAI-gene was attached to a seed-specific promoter and the
chimeric gene was introduced into garden peas (Pisum sativum L). The
seeds of the resulting transgenic peas showed a high degree of
resistance to the bruchids cowpea weevil and Azuki bean weevil. This
achievement may be particularly important in developing countries, not
only because of the large amount of post-harvest losses, but also
because of the importance of legumes such as peas as a source of
protein. [14)
3.1.1.3 Lectins
Lectins are proteins capable of binding to carbohydrate moieties of complex
carbohydrates without altering the structure of the carbohydrate.
They were discovered about 100 years ago when the observation was
made that extracts of beans agglutinate red blood cells. Further
research showed that many plants contain lectins. They are predominantly
found in seeds where they can comprise up to two percent of the total
protein. The actual function of lectins in plants is not clearly
understood, but they are believed to function as a defense mechanism
against a variety of fungal and bacterial pathogens. Deleterious
effects were found e.g. against weevil larvae and European corn borer.
The mechanism of toxicity is unknown but probably involves the binding
of lectin to insect midgut cells and disrupting of cell functions or
inhibition of nutrient uptake.[16]
The lectin wheat germ agglutinin has been introduced into maize to achieve
insect resistance and field trials have been carried out. Hilder et al.
transformed tobacco with a snowdrop (Galanthus nivalis) lectin. They
reported enhanced resistance of the transgenic tobacco against the
sap-sucking peach potato aphid (Myzus persicae). However, no
commercially acceptable level of protection was reached.[17]
Resistance against insects feeding on phloem sap may be enhanced by
using phloem-specific promoters such as the rice sucrose synthase
(RSs1) promoter. [15]
3.1.2 Protection against Viral Infections
Viruses cannot be controlled by chemical means. As viruses can cause
substantial losses in many crops, they are indirectly controlled by
spraying chemicals against virus vectors (mainly insects), by using
certified virus-free material, or by eradicating infected plants. As
these measures are not overly successful, the engineering of virus
resistance into plants is expected to provide a direct and more
efficient control.
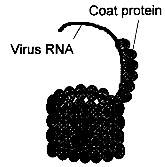
Fig. 3.1: Model of tobacco mosaic virus (TMV) assembly showing the virus
RNA surrounded by coat proteins. TMV is about 18 nm in diameter and
300 nm long. [6]
Viruses are genetic elements much smaller than e.g. bacterial cells. They
do not have an own matabolism and multiply only inside living host cells
using the cell metabolic machinery. Viruses consist of either a DNA or
RNA genome surrounded by a coat protein (see fig. 3.1), and occasionally
other material. When a virus multiplies, the viral genome is released from
the coat into the host cell, where the host metabolism starts produtcion of
viral proteins and replication of viral genes, finally resulting in the release of
new viruses. About 80% of pathogenic plant viruses have genomes of plus
strand single-stranded RNA which may be directly used as mRNA, but also
single- or double-stranded DNA viruses cause significatn losses to crops.
Most genetic engineering strategies against viruses are based on the
introduction of a virus-derived gene sequence into the genome of the host
plant. The products of these sequences (mRNA and proteins) interfere with
specific stages in the viral infection cycle, such as virus replication or
spread, thereby resulting in a virus resistat plant. Resistance mediated by
expression of a viral coat protein is the most advanced approach and has
already led to resistant varieties which are expected on the market soon.
Some other strategies also confer a high degree of resistance, e.g.
replicase-mediated protection, but no commercial products have been
developed ye using these methods.
3.1.2.1 Coat Protein-Mediated Protection
Biological Basis
The genome of plant viruses encodes a coat protein that protects the viral
nucleic acid during the transfer from plant to plant and can determine
the specificity of the vector. In coat protein-mediated protection
(CPMP), a gene for a viral coat protein is introduced into the genome
of the plant. The concept of CPMP has been derived from the observation
of cross protection: by preinfection with a mild symptomless virus strain,
plants are protected against a related but severely damaging strain.
The first report on CPMP was published in 1986, when the Tobacco Mosaic
Virus (TMV) coat protein gene was introduced into the genome of
tobacco plants. Transgenic plants expressing high levels of coat
protein showed a delay in disease development upon infection with
TMV.[9]
Typically, CPMP is achieved by transforming plants with a functional sense
coat protein gene under control of a Cauliflower Mosaic Virus (CaMV)
35S promoter that mediates strong constitutive transcription in many
cell types. CPMP works against the virus strain from which the coat
protein gene originates and against related viruses. The mechanism of
this resistance is not fully understood. The degree of resistance was
often correlated with the amount of intact coat protein expressed in
transgenic plants and resistance could be overcome by high concentrations
of virus inoculum. Several lines of evidence suggest that the coat
protein produced. by the plant impedes the uncoating of the viral RNA
at the beginning of the infection cycle. In some cases, resistance was
found in plants expressing only low levels of coat protein, in plants
expressing defective coat proteins, or in plants expressing only coat
protein mRNA which was not translated into protein. In these examples,
resistance may be mediated by the coat protein mRNA.[18]
State of Development
So far, CPMP is the only method for genetically engineered resistance which
has the potential for agronomic use. CPMP has been demonstrated for
more than 20 RNA viruses, but no effect on DNA viruses has yet been
reported. Tobacco has been used as a model system to study CPMP, but
resistance has now also been engineered into important field crops
such as potato, tomato, squash, alfalfa, and has also been extended
to cereals.[9]
The efficiency of CPMP has been tested in field trials with several plant
species (see table 3.2). The first field trial was conducted in 1987
with transgenic tomato plants. After mechanical inoculation with tobacco
mosaic virus (TMV), at the time of fruit harvest only 5 % of the
transgenic plants expressing the TMV coat protein had developed
disease symptoms, compared to 99% of the control plants. The fruit
yield was equal to that of non-infected plants.[9] Other
trials have shown similarly high degrees of resistance, demonstrating
the usefulness of this approach. The US seed company Asgrow has
developed a transgenic squash variety resistant to zucchini yellow
mosaic virus and watermelon virus II using coat protein genes of the
two viruses. Asgrow has obtained market approval and commercial sales
are expected soon (see chapter 4.4).
Crop |
Resistance against |
Crop |
Resistance against |
Alfalfa |
AIMV, CMV, TMV, PRV |
Papaya |
PRV |
Barley |
BYDV |
Petunia |
CMV |
Beet |
BNYVV |
Plum tree |
PRV |
Cantaloupe |
CMV, WMV2, ZYMV, TEV |
Potato |
PVX, PVS, PVY, PLRV, BYDV, BYMV |
Cucumber |
CMV, ZYMV |
Rice |
RSV |
Gladious |
BYMV |
Soybean |
SMV |
Lettuce |
TSMV |
Squash |
ZYMV, WMV2, CMV, SMV, PRV |
Maize |
MDMV, MCDV, CMV, TMV |
Tobacco |
TMV, TRV, TEV, AIMV, TSV,CMV, ArMV, TSWV |
Melon |
CMV, PRSV, ZYMV, WMV2, PRV |
Tomato |
TMV, AIMV,TSWV, TYLCV, PVX, PVY, PLRV |
Oilseed rape |
TMV, CMV |
Peanut |
TSWV |
|
|
Watermelon |
WMV2, ZYMV |
Table 3.3: Field tested crops with a coat protein-mediated protection against
viruses.
Sources: APHIS Biomonitoring database [19], GIBiP database [20]
Abbreviations: AIMV alfalfa mosaic virus, ArMV arabis mosaic virus, BNYVV
beet necrotic yellow vein virus, BYDV bailey yellow dwarf virus, BYMV
bean yellow mosaic virus, CMV cucumber mosaic virus, MDMV maize dwarf
mosaic virus, PLRV potato leafroll virus, PRSV potato ringspot virus,
PRV papaya ringspot virus, PVS potato virus S, PVX potato virus X,
PVY potato virus Y, RSV rice stripe virus, SMV soybean mosaic virus,
TEV tobacco etch virus, TMV tobacco mosaic virus, TRV tobacco rattle
virus, TSV tobacco streak virus, TSWV tomato spotted wilt virus,
TYLCV tomato yellow leaf curl virus, WMV2 watermelon mosaic virus II,
ZYMV zucchini yellow mosaic virus.
3.1.2.2 Replicase-Mediated Protection
Biological Basis
As an alternative to the use of genes encoding structural proteins like
coat protein genes, the introduction of sequences coding for non-
structural viral proteins has been exploited to create virus resistant
plants. There are several reports now describing how expression of a
viral RNA-polymerase in transgenic plants leads to resistance. The
viral RNA-dependent RNA-polymerase, also called replicase, is used by
the virus to generate new RNA copies of the viral RNA genome. The
first report was published in 1990, when it was discovered that
transgenic tobacco plants expressing part of the replicase gene of a
TW were highly resistant to this virus and a closely related strain.
The mechanisms conferring resistance are not clear. Resistance was
brought about both by replicase genes which coded for functional
proteins and by mutated genes which lead to production of non-functional
protein or no protein at all. One explanation of resistance is based
on the observation that overproduction of one component of the
transcription complex (in this case the replicase) can lead to
inhibition of transcription. If defective replicase is expressed,
resistance may result from the effect of the dysfunctional protein
which dominates the functional viral proteins. The efficacy of both
proposed mechanisms should increase with enhanced expression of the
transgene. However, these explanations are in contrast with several
experiments where the highest level of resistance has been observed
in the plants with the lowest expression rate of the transgene, an
effect which has also been found in coat protein-mediated resistance.
In these cases, the resistance may be mediated by mRNA, as proposed
by Baulcombe in.[21]
State of Development
In the last five years, at least eight examples of replicase-mediated
resistance in model plants have been published, including resistance
against tobacco mosaic virus, pea early browning virus, cucumber mosaic
virus, potato viruses X and Y, and cymbidium ringspot virus. Though
apparently no field trials have been carried out, the extreme level
of resistance observed in some experiments makes it likely that this
approach will be useful in the field. A limitation may be the strain
specificity of the effect, which could cause problems in genetically
heterogenous viral field isolates.[21]
3.1.2.3 Antisense RNA-Mediated Resistance
Biological Basis
Antisense RNA is RNA complementary to mRNA of a particular gene. Antisense
RNAs were initially recognized in bacteria as naturally occurring
mechanisms of gene expression. Antisense strategies are increasingly
used as a means of down regulating specific genes. The most famous
application to date is the FlavrSavrTM tomato with an
extended shelf life which was the first transgenic food available on
market. The extended shelf life is achieved by down regulating the
polygalacturonase (PG) gene responsible for the softening of the
tomato using an antisense gene of the PG gene. Expression of antisense
RNA in transgenic plants is achieved by introducing the noncoding
strand of a gene together with an appropriate promoter into the plant
genome. The mode of action of antisense RNA is not clearly understood.
The antisense RNA is thought to form a duplex with the target viral
RNA by hybridization. The duplexes formed may stimulate degradation
of the mRNA by RNAses or could prevent binding of the ribosome,
thereby impeding translation into proteins (see figure 3.2).
[22]
State of Development
Research with antisense genes used for virus resistance has been
predominantly conducted with tobacco and potato. The antisense
approach was successful in several cases, e.g. in transgenic Russet
Burbank potato against potato leafroll virus (PLRV). In this case,
sense and antisense constructs against viral coat protein were equally
effective. Examples of successful induction of resistance also include
tomato golden mosaic virus (TGMV), a single stranded DNA virus which
replicates in the nucleus. In several other instances, the protection
was less effective, e.g. in tobacco expressing antisense coat protein
genes of PVX. [22]
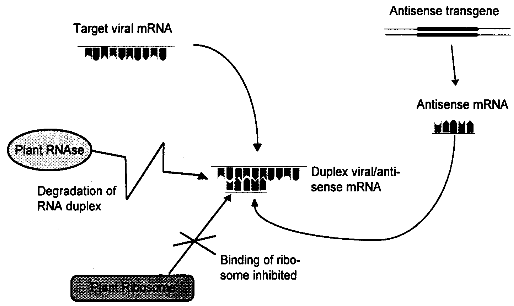
Fig. 3.2: Possible mechanisms of antisense gene action. The antisense
RNA is thought to hybridize with the target viral sequences, thereby
forming a RNA duplex. This duplex may then be degraded quickly by plant
RNAses (enzymes which break down RNA), or the attachment of the antisense
RNA may prevent binding of the ribosomes (ribosomes are key components of
the protein-sythesizing machinery of the cell). [22]
3.1.2.4 Satellite RNA-Mediated Disease Attenuation
Biological Basis
Satellite RNAs are small extragenomic RNA molecules found in some plant
viruses. They cannot replicate individually and depend on a helper
virus for replication. They have no sequence similarity with their
helper virus, and are thus not directly derived from the helper virus
genome. Some satellite RNAs are known to attenuate the symptoms of
the helper virus. For example, in China, pepper plants are
prophylactically inoculated with a cucumber mosaic virus (CMV) strain
which contains satellite RNA. These plants develop only mild symptoms
and are protected against infection with a more harmful CW strain not
containing the satellite. Transgenic plants expressing satellite RNA
were shown to be protected against the severe effects of their helper
virus. As a mechanism for satellite RNA-mediated disease attenuation,
competition with the viral genomic RNA for a limiting amount of
replicase enzyme has been proposed, but other mechanisms seem to
contribute to the disease attenuation as well.[9]
State of Development
The most widely studied satellite RNAs are those associated with cucumber
mosaic virus (CMV). Several groups have shown that transgenic plants
expressing CMV satellite RNA are tolerant to CMV. Transgenic plants
showed almost no symptoms after infection with CMV. Also tobacco plants
expressing tobacco ringspot virus (TobRSV) satellite RNA were tolerant
to TobRSV.[9] Few researchers envisage field testing of
transgenic plants due to the possibilities of producing deleterious
satellite RNA (see chapter 4.1.4.2). As mentioned before, cross
protection with a CMV strain attenuated by a natural satellite RNA
has been shown in the field, not only at a large scale in China, but
also at smaller scale in U.S. and Italy. Large-scale tests of transgenic
plants expressing satellite RNA are being carried out in China.
[23]
3.1.2.5 Defective Interfering RNA/DNA Protection
Defective interfering (DI) particles are deletion mutants of genomic viral
sequences which depend on their parent virus for replication. DI RNAs
have frequently been described in animal virus systems but, so far,
only rarely in plant virus systems. Like satellite RNAs, DI particles
can attenuate the disease symptoms of their parent virus by interfering
with its replication, as found for tomato bushy stunt virus (TBSV).
As an alternative to the introduction of naturally occurring DI molecules,
transformation with artificially constructed Dis have been described.
Deletion mutants of several viral RNAs were shown to reduce the
replication of the respective parent viruses.[9]
3.1.2.6 Ribozyme-Mediated Protection
Ribozymes are RNA molecules catalyzing RNA cleavage reactions. Mostly, the
cleavage site consists of a consensus structure, called the
"hammerhead" motive. The nucleotide region directing the catalysis of
the cleavage reaction could be separated from the region where the
cleavage occurs and the recognition of the target could be modified
by changing the nucleotide sequence of the regions flanking the cleavage
site. Thus, ribozymes could be designed which cleave viral sequences.
Transgenic plants could express a ribozyme which cleaves viral
nucleotides. Whether ribozymes will be active in stably transformed
plants, and by which expression strategy a sufficient amount of ribozyme
can be produced to achieve a reduction in target gene expression
remains to be investigated.[9]
3.1.3 Resistance to Fungal Pathogens
plants exhibit natural resistance to fungal attack, and disease is the
exception rather than the rule. However, the exceptions can be costly
and even devastating. Fungal disease remains one of the major factors
limiting crop productivity worldwide, with often huge losses set against
massive cash inputs for pesticide treatments, exemplified by an
estimated cost to the wheat farmers of US$100 million per annum in
Europe alone. [24] The total market for fungicides amounts
about US$ 5.6 billion world-wide (1991).[1] Hence, there is
great interest in the development of novel strategies for protecting
crop plants against fungal diseases, to complement traditional plant
breeding efforts and to reduce the expense and possible environmental
costs of reliance on conventional agrochemical treatments.
[24]
Although several promising approaches exist, no transgenic varieties with
fungal resistance are expected to approach market introduction in the
near future. Strategies for obtaining plants resistant to fungi are
largely based on natural plant responses to pathogen attack. Therefore,
the following chapter starts with a short introduction into the
principles of natural plant disease resistance. Although this part
deals with resistance to fungi, many of the methods described may also
be used to achieve enhanced resistance to other pathogens, e.g.
viruses, bacteria, and nematodes.
3.1.3.1 Natural Plant Disease Resistance
Plants, like animals, are continually exposed to pathogen attack. Plant-
pathogenic organisms are diverse and include viruses, bacteria, fungi,
nematodes and protozoa. These pathogens usually live within the plant
cell or in the intercellular space. Because plants lack a circulatory
system and antibodies, they have evolved a defense system that is
distinct from the vertebrate immune system. In contrast to animal cells,
each plant cell is capable of defending itself by a combination of
different defense reactions. Resistance to a pathogen is often correlated
with a "hypersensitive response", induced cell death in the host plant
at the site of infection. The hypersensitive response (HR) involves
generation of active oxygen species, ion fluxes across membranes,
cross-linking and strengthening of plant cell wall, production of
antimicrobial compounds (phytoalexins) and induction of pathogenesis-
related (PR) proteins such as chitinases and glucanases. Though the
mechanisms are unknown, HR is thought to be responsible for the
limitation of pathogen growth. The HR is hypothesized to trigger a
subsequent response to pathogen attack, referred to as systemic
acquired resistance, that acts throughout the plant and not only at
the site of infection. Systemic acquired resistance reduces the
severity of disease caused by all classes of pathogens, including
normally virulent pathogens. [25]
Whether a plant is susceptible or resistant to a specific pathogen often
depends on its ability to induce HR. Induction of HR follows recognition
of specific signal molecules (elicitors) produced by the pathogen.
These elicitors are directly or indirectly coded for by so-called avr
(for avirulence) genes of the pathogen. The receptors of the plant
cell which recognize the elicitors and (indirectly) initiate the HR
are coded for by R (for resistance) genes. Therefore, a plant is only
protected against pathogens which produce a specific elicitor that is
recognized by a specific plant receptor as shown in figure 3.3.
[25]
In conventional plant breeding, plants with resistance (R) genes against
important pathogens have been selected according to their phenotypic
traits and the resistance has been introduced into other varieties by
hybridization. Unfortunately, resistance of a new variety conferred
by a single resistance gene is often overcome after only a few years
by the development of a new pathogen race, and the combination of
several resistance genes by conventional breeding is even more time-
consuming than introduction of a single R gene.
Today, the growing understanding of the mechanisms leading to plant disease
resistance is increasingly used for plant genetic engineering. Recently,
the first R genes have been identified and described in plants. These
include R genes from Arabidopsis sp. (resistance to the bacterium
Pseudomonas syringae), tobacco (resistance to tobacco mosaic virus and
to P. syringae), tomato (resistance to the leaf fungal pathogen
Cladosporium fulvum) and flax (resistance to a leaf rust fungal race).
[26] The identification of such R genes will not only
facilitate the understanding of plant disease resistance, but also
makes possible the direct introduction of R genes in different plant
varieties using genetic engineering. By combining different R genes
responsive to the same pathogen in one plant, resistant varieties
with a more durable resistance could be produced.
Genes acting at other levels of the plant defense system have already been
introduced into plants in order to increase resistance against fungi
and other pathogens, as described in the following chapters.
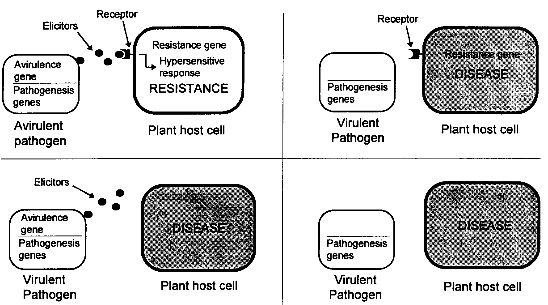
Fig. 3.3: Gene-for-gene interactions in host-pathogen systems. The
plant cell can only induce the hypersensitive (HR) if the pathogen produces
a signal molecule - coded for by an avirulence gene - which is recognized
by a plant receptor, coded for by a resistance gene (upper left panel).
If either avirulence gene or corresponding resistance gene or both are
lacking (other panels), no HR is induced and disease developes. Adapted
from [25]
3.1.3.2 Chitinases and Glucanases
Chitin and β-1,3-glucans are major structural polysaccharides of the
cell walls of many fungi. The breakdown of these components by plant
endochitinases and β-1,3-glucanases, both belonging to the group
of the pathogenesis-related (PR) proteins of plants which are induced
upon pathogen attack, is thought to inhibit fungal growth. Several
types of endochitinases and β-1,3-glucanases have been described.
A combination of a bean endochitinase gene with the promoter of the
CaMV 35S gene conferring high constitutive expression in a wide
variety of plant cells was introduced into tobacco. For evaluation,
the transgenic tobacco was grown in presence of Rhizoctonia solani,
a soilborne fungus that infects numerous plant species. Seedling
mortality or the loss of root fresh weight was clearly reduced compared
to control plants, depending on the amount of bean chitinase expressed.
[27] Similar effects were found with other 35S-chitinase
constructs, but in other cases this approach did not reveal a substantial
increase of resistance to chitin-containing fungi.[9]
Recently, a rice chitinase gene has been introduced into protoplasts of
an Indica rice variety using polyethyleneglycol-mediated transformation,
and fertile plants have been regenerated. This was one of the first
reports of regeneration of the important Indica rice, as these varieties
have been particularly difficult to regenerate. Transgenic plants
expressing the chitinase showed increased resistance to the fungal
sheath blight pathogen Rhizoctonia solani. [28]
In another recently published paper, the combined action of chitinase and
glucanase was evaluated. The rice RCH10 chitinase and the alfalfa AGLU1
glucanase under control of CaMV 35S promoters were introduced separately
into tobacco. The transgenic lines expressing one of the two hydrolytic
enzymes were then selfed, and the homozygous progeny lines were crossed
with each others. The resulting lines were infected with the fungal
pathogen Cercospora nicotianae. Evaluation of disease development showed
that the combination of chitinase and glucanase gave the best protection,
with only weak symptoms after substantial delay (see figure 3.4).
[29]
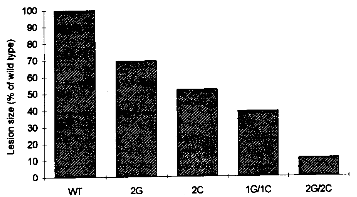
Fig. 3.4: GProtective effects of glucanases and chitinases in
transgenic tobacco. WT wild-type, 2G homozygous for glucanases,
2C homozygous for chitinases, 1G/1C heterozygous for both enzymes,
2G/2C homozygous for both enzymes. [29]
The finding that combination of genes which alone have only limited effect
results in significant protection is promising. Similar results have
been found in other experiments (see below). Moreover, combinatorial
use of antimicrobial genes may also reduce the breakdown of resistance
as a result of pathogen mutation.[29]
3.1.3.3 Ribosome-inactivating Proteins
Ribosome-inactivating proteins (RIPs) are plant proteins which inhibit
protein synthesis in target cells by cleavage of 28 S RNA. RIPs do not
inactivate "self' ribosomes, but show activity towards ribosomes from
distantly related species including fungi. Some ribosomes are among
the most potent natural toxins, the best known of which is ricin.
[24] Purified barley RIP inhibits fungal growth in vitro,
and its effect is synergistically enhanced by chitinases and
β-1,3-glucanases. These characteristics were used to improve
resistance to Rhizoctonia solani in transgenic tobacco. A barley RIP
was put under control of the potato wun1 promoter which mediates wound-
and pathogen-inducible transcription in the epidermis of leaves, stems
and roots from tobacco. Primary transformed regenerates and R1 progeny
grew more vigorously in soil inoculated with Rhizoctonia solani than
non-transformed control plants. [9]
3.1.3.4 Phytoalexins
In several host/pathogen systems a correlation has been found between the
concentration of phytoalexins (antimicrobial compounds synthesized by
plants) and resistance to specific pathogens. In Vitis vinifera and
Picea sitchensis, stilbenes are involved in protection against fungal
challenge. Most plants, for example tobacco, contain the precursor for
the formation of stilbenes but lack the enzyme stilbene synthase. After
transfer of a stilbene synthase gene from grapevine to tobacco, transgenic
plants expressed the foreign gene in response to treatment with fungal
elicitor or infection with the broad range fungal pathogen Botrytis
cinerea. This work supports the assumption that at least in some
host/pathogen systems the synthesis of phytoalexins plays a crucial
role for the defense of the host plant. [9]
3.1.3.5 Other Antifungal Proteins
A large number of other proteins with inhibitory effects on the growth of
fungi in vitro'have been identified from plants. Among these are osmotin,
a vacuolar protein from tobacco produced in response to salt stress,
and small, basic, cysteine-rich proteins of various origins. Also some
microorganisms produce such antifungal peptides. [9]
These proteins will be tested - alone or in combination - for their
effect against fungi in transgenic plants and at least some of them
are likely to enhance resistance in transgenic crop plants.
3.1.3.6 Hypersensitive Cell Death
In the past, crossing-in of race-specific resistance was frequently applied
in breeding programs to protect novel varieties against fungal and
other diseases, as mentioned before. However, this procedure provides
protection only against a limited number of pathogen races and large-
scale growth of new varieties led to the rapid selection of new
virulent races and epidemic spread in monocultures. Of course, the
same problems could also arise if transgenic plants carrying race-
specific resistance genes from other cultivars or other species were
grown in the field. Different strategies to obtain durable resistance
to a wide range of pathogens using artificial generation of programmed
cell death are being pursued.
One of the most advanced was proposed by Strittmatter et al... [9]
A promoter fragment of the potato prp11 gene, which mediates rapid and
local transcriptional activation selectively after fungal infection,
has been combined with the barnase gene from Bacillus amyloliquefaciens
encoding a highly cytotoxic RNase. The resulting chimeric construct was
transformed into potato. Rapid synthesis of this RNase in the vicinity
of infection sites should initiate necrosis of host cells during early
stages of compatible interactions and, therefore, restrict the growth
and propagation of biotrophic pathogenic fungi also in this type of
interaction, analogous to the naturally occurring hypersensitive cell
death in incompatible interactions. Transgenic plants were simultaneously
transformed with a chimeric construct which constitutively produces
barstar the specific barnase inhibitor from Bacillus amyloliquefaciens.
The authors expect, that only in the close vicinity of infection sites
the level of cytotoxic barnase will exceed the inhibitor barstar,
resulting in strictly localized cell death after infection.
3.1.3.7 Systemic Acquired Resistance
As mentioned in chapter 3.1.3.1, a hypersensitive response is often followed
by a subsequent response called systemic acquired resistance (SAR).
SAR acts throughout the plant and protects it against a wide range of
pathogens. Establishment of SAR is correlated with the coordinate
expression of several groups of genes, so-called pathogenesis-related
(PR) proteins. PR-1a is the most highly expressed of these proteins.
The gene coding for PR-1a was brought under control of an enhanced
35S promoter and transformed into tobacco. Transgenic tobacco lines
showed constitutive high-level expression of the PR-1a protein. This
resulted in significant tolerance to infection by the two fungal
pathogens Peronospora tabacina and Phytophthora parasitica var.
nicotianae. [30]
Although the function of PR-1a protein and other PR proteins is not known
yet, high-level expression of such proteins may be useful in creating
crops with increased resistance to fungal and other diseases.
3.1.4 Resistance to Bacterial Pathogens
Bacterial diseases are a persistent problem, with no satisfactory alternative
to copper sulfate treatment.[24] As these treatments cause
environmental problems, there is a great interest in the development
of novel strategies. While scientific progress has been im- pressive,
examples of agronomically significant resistance to plant-pathogenic
bacteria are still scarce.[4]
3.1.4.1 Lysozyme-Mediated Resistance
Lysozymes, which catalyze the hydrolytic cleavage of bacterial cell wall
murein, have been detected in many plant species. As the biochemical
and molecular characterization of plant lysozymes and the corresponding
genes is still in its infancy, lysozyme genes from other sources have
been used for genetic engineering. The expression of lysozyme genes
from hen egg white or bacteriophage T4 was achieved in tobacco and
potato. One group found an increased resistance of tuber slices from
transgenic potato lines to the pathogenic soil bacterium Erwinia
carotovora spp. atroseptica.[9]
3.1.4.2 Cecropins
Cecropins are a family of proteins which form an important component of the
immune response of diverse insects. They exhibit antibacterial activity
against several Gram-positive and Gram-negative bacteria in vitro,
apparently by forming ion channels in the bacterial membrane leading
to leakage of cell components and ultimately cell death.
[31]
A gene coding for cecropin B from the giant silkmoth Hyalophora cecropia
was introduced into tobacco Although considerable amounts of
cecropin-mRNA were present in transgenic plants, no cecropin B
protein could be detected. As rapid degradation of cecropin B was
shown in crude plant extracts, the undetectable level of cecropin B
in transgenic tobacco is thought to result from degradation of the
protein by plant-endogenous proteases. Transgenic plants therefore
showed no resistance against the bacteria Pseudomonas solanacearum
and Pseudomonas syringae, which are highly susceptible to cecropin
B in Vitro.[31]
In another approach, substitution analogs of cecropin were produced. In
these cecropin analogs, the amino acid sequence was changed but
hydrophobic properties and charge density was conserved. One of
these substitution analog called Shiva-I with 46 % homology in amino
acid sequence was found to have a more potent lytic activity than
another cecropin analog with 95 % homology. The gene sequence for
Shiva-I was introduced into tobacco. Transgenic tobacco seedlings
exhibited delayed wilt symptoms, reduced disease severity and reduced
mortality after infection with a highly virulent strain of Pseudomonas
solanacearum. [32]
3.1.4.3 Activation of Plant Defense Mechanisms
Phytoalexins are low molecular weight antimicrobial compounds which are
synthesized in plants in response to pathogen attack (see chapter
3.1.3.1). The synthesis of phytoalexins is in most cases based on
complex biosynthetic pathways which are not accessible for genetic
engineering yet. An exception are thionins, small cysteine-rich
polypeptides which were found in endosperm and leaves of cereals.
They were shown to exert antimicrobial activity in vitro. One group
obtained high level synthesis of functional thionin in transgenic
tobacco leaves transformed with a barley a-thionin gene under control
of the CaMV 35S promoter. After inoculation with Pseudomonas syringae
pv. tabaci or Pseudomonas syringae pv. syringae, the number of necrotic
lesions and the severity of disease symptoms were reduced in leaves
of transgenic plants compared to control plants. The level of
resistance coincided with the level of thionin expression.
[9]
3.1.4.4 Detoxification of Pathotoxins and Alteration of Target
Enzymes
Pathogens that produce phytotoxins causing disease symptoms usually have
the capacity to metabolize, i.e. detoxify these compounds.[4]
For instance, the dipeptide tabtoxin is thought to be responsible
for chlorosis during wildfire disease on tobacco caused by Pseudomonas
syringae pv. tabaci. The pathogen protects itself against the toxin
by expression of the tabtoxin resistance gene, ttr, which encodes an
enzyme that acetylates tabtoxin. Transgenic tobacco plants
constitutively expressing the ttr gene under control of the CaMV 35S
promoter did not produce the chlorotic halo typical for wildfire
disease on non-transgenic plants.[9]
In a comparable strategy, protection against the damaging effects of a
bacterial toxin was obtained by transformation of plants with a
pathogen-derived enzyme which is unsusceptible to the toxin. Transgenic
tobacco plants harboring a gene construct derived from Pseudomonas
syringae pv. phaseolicola expressed an ornithine carbomyl transferase
which is insensitive to the phaseolotoxin produced by Pseudomonas
syringae pv. phaseolicola. Progeny of these plants displayed a reduction
in disease symptoms compared to control plants.[9]
3.2 Protection Mediated by Transgenic Microorganisms
In contrast to the plant protection approaches based on transgenic plants
discussed above, the following chapters describe methods which make
use of genetically altered viruses and bacteria, mostly to achieve
plant protection against insect pests. Mainly insect-pathogenic
baculoviruses and bacteria other than Bacillus thuringiensis expressing
Bt toxin have been developed.
3.2.1 Transgenic Baculoviruses
Biological Basis
Baculoviruses are pathogens of insects that infect predominantly
holometabolous insects. Almost all Lepidopterans, which include many
of the world's most serious pests, are susceptible to at least one of
the more than 500 baculovirus species. The baculoviruses were first
studied for use against forest pests in the 1930s and were used until
the advent of chemical pesticides in 1960. The virus particles are
generally applied on the foliage by spray. The viruses have no contact
action and must therefore be ingested by larvae, thus good coverage
of plants is required. Of particular interest for insect pest
management is Autographa californica nuclear polyhedrosis virus (AcNPV),
originally isolated from the alfalfa looper, and the Bombyx mori
nuclear polyhedrosis virus (BmNPV).2
The DNA of a baculovirus is enclosed within a so-called nucleocapsid,
about 50 by 300 nm in size, which again is enclosed within an occlusion
body composed of the protein polyhedrin. These occlusion bodies, each
of them containing several nucleocapsids, are referred to as polyhedra.
The polyhedra protect the virions in the environment. After ingestion
of the polyhedra by an insect, the polyhedrin is dissolved and the
nucleocapsids fuse to the midgut cells and migrate to the nucleus,
where replication takes place. The produced progeny nucleocapsids
spread and infect other cells and tissues, resulting in infection
throughout the insect. Nucleocapsids may then be enclosed in new
polyhedra synthesized in the nucleus. Before the death of the host,
the polyhedra can account for up to 30% of the insect dry weight.
The insect may continue to feed for several weeks before death from
viral infection. The cadaver filled with virus ruptures easily,
releasing millions of polyhedra onto surrounding foliage and soil.
[33]
The fact that baculoviruses take weeks for killing their hosts is one of
the most important disadvantages associated with the use of
baculoviruses as insecticides. It has restricted their use to crops
capable of sustaining some damage without too much economic loss. In
order to decrease the killing time of the baculoviruses and the crop
damage, genes of different origins have been introduced into AcNPV,
including genes encoding insect hormones, insect-specific toxins and
insect enzymes.[33]
State of development
Field trials with genetically engineered baculoviruses were carried out in
England in 1986, 1987 and 1988. The trials examined the persistence
of genetically altered AcNPV and were among the first deliberate
releases of transgenic organisms.[34] The first recombinant
baculovirus with an enhanced insecticidal effect was developed in 1989.
The gene of a diuretic hormone which plays an important role in the
regulation of the water balance in the insect Manduca sexta (tobacco
hornworm) was incorporated into Bombyx mori nuclear polyhedrosis virus
(BmNPV). Bombyx mori larvae infected with the transgenic virus died
20% more quickly than larvae infected with wild-type BmNPV. The expression
of a toxin of the North African scorpion Androctonus australis in
AcNPV resulted in a 50 % reduction of feeding damage, and lethal time
was reduced by 25 % compared to wild-type viruses. A toxin from the
straw itch mite Pyemotes tritici also introduced into AcNPV to enhance
its insecticidal activity reduced time to kill by 30-40%. The titre
of juvenile hormone (JH) in haemolymph determines the course of larval
development. A reduction in JH titre controlled by juvenile hormone
esterase (JHE) leads to cessation of feeding before a moult and
initiates metamorphosis. By expression of modified JHEs in AcNPV,
insecticidal viruses with lethal times more than 30 % lower than with
wild-type viruses were generated [33]
Currently, no commercial application of genetically engineered insect
viruses is in sight, but their use is thought to have considerable
potential. The company American Cyanamid e.g. will conduct field trials
with transgenic baculoviruses expressing scorpion toxin in the USA.
[35]
2 The viruses are sometimes wrongly called "Autographa
californica' or "Bombyx mori" only. These are the scientific names
of the insects from which the viruses were first isolated.
3.2.2 Transgenic Bacteria
3.2.2.1 Inactivated Pseudomonas Fluorescens for Bt Toxin
Delivery
Biological Basis
In this approach, a non-pathogenic strain of Pseudomonas fluorescens
bacteria is used as host for the Bacillus thuringiensis (Bt) toxin
(see chapter 3.1.1.1). In an attempt to improve the foliar persistence
of Bt insecticidal activity, a delivery system based on recombinant
P. fluorescens expressing Bt toxin which are killed by chemicals prior
to field release, has been developed by Mycogen Corporation
(San Diego, California). When transformed cells are grown in culture,
a typical Bt δ-endotoxin crystal is formed within the cell.
While still in the fermentation tank, the P. fluorescens cells are
chemically treated in order to kill the cells and cause the cell wall
to become more rigid through cross-linking of cell wall components.
The cell wall of the dead bacteria now serves as a protective microcapsule
for the enclosed δ-endotoxin. This Bt toxin delivery system has
been called CellCap ® by Mycogen. [36]
State of Development
Since no living transgenic microorganisms are released into the environment,
this approach has the advantage of relative freedom from environmental
and safety concerns associated with field releases of living transgenic
organisms. The encapsulated Mycogen product with a lepidopteran active
δ-endotoxin was the first recombinant product approved for
outdoor testing. Field experiments conducted during 1988 suggested
that a foliar application of this engineered microbe protected cabbage
from lepidopteran pests for 7 days, whereas insecticidal activity from
traditional Bt sprays dissipated by 3 days post-application. This
resulted in better insect control, and therefore higher yields. Similar
tests have been conducted with engineered microbes targeted against
the Colorado potato beetle on potatoes. It was shown that the protected
δ-endotoxin could be applied at two thirds the toxin rate and
still maintain higher levels of insect control than the full rate
delivered by Bacillus thuringiensis.
U. S. federal registrations for the first two genetically engineered
bioinsecticides using Mycogen's CellCape® encapsulation
system, MVP® (lepidopteran-active biotoxin) and
M-TrakTM (coleopteran-active biotoxin) were received in
1991 from the Environmental Protection Agency. Commercial sales began
that year. [36]
3.2.2.2 Bacterial Endophytes Expressing Bacillus Thuringiensis
Toxins
Biological Basis
The term endophyte is used for plant-associated microorganisms that live
within their host plants. Genetic engineering of nonpathogenic
endophytes presents an opportunity for delivery of biopesticides in
the plant, i. e. the production of the pesticidal agent within host
plant tissues without direct genetic manipulation of the host plant.
Most approaches make use of Bacillus thuringiensis (Bt) toxin. The
major drawback in using Bacillus thuringiensis directly as a
biopesticide lies in the fast degradation of the Bt toxin after
spraying the bacteria with the Bt toxin crystal outside the spore
(see chapter 3.1.1.). A systemic delivery system using bacteria living
within the plant would protect the Bt toxin from degradation,
providing consistent levels of protection throughout the growing
season by the presence of the active ingredient produced by the
endophyte. Areas difficult to reach with chemical sprays (e.g., the
inside of stalks) would be better protected from plant-feeding insects
by endophytes expressing Bt toxin. A number of plant-associated
bacteria have been transformed with Bt toxin genes, including Pseudomonas
fluorescens, Pseudomonas cepacia, Rhizobium meliloti and Rhizobium
leguminosarum. [8] In the following, the use of transgenic
Clavibacter as biopesticide will be described in more detail, as
this application is one of the most advanced in this field.
[37]
State of Development
The Gram-negative bacterium Clavibacter xyli ssp. cynodontis (Cxc) is a
common endophyte living in the xylem of Bermudagrass (Cynodon dactylon)
and has been found in this host in the United States, Japan, Taiwan,
and France. It has also been found in a few weed species, and it can
colonize maize, rice, sorghum, oats, white millet and sudangrass
through artificial inoculation. The gene coding for the CrylA(c)
protein from Bt ssp kurstaki was inserted into the chromosome of a
wild-type Cxc. Unlike Bt, transgenic Cxc do not release the Bt toxin.
Instead, the cell must be digested by the insect in order to release
the active ingredient in the gut. The observed effects are similar
to those observed in larvae ingesting Bt (see chapter 3.1.1.). Field
studies have demonstrated that a wide range of commercial field and
sweet maize hybrids can be inoculated successfully, with 80-100 % of
the inoculated seeds producing vigorous, endophyte colonized plants.
The European corn borer (ECB), Ostrinia nubilalis, is cosmopolitan
in its distribution and a significant pest in maize. Maize plants
inoculated with recombinant Cxc; and artificially infested with ECB
larvae sustained up to 80 % less damage than plants inoculated with
either wild type Cxc or uninoculated plants. C= was the first
recombinant microorganism expressing Bt toxin which has been approved
for field testing [37]
|