Sie sind hier: Landwirtschaft > Transgene Pflanzen > Genet. Pflanzenschutz > Zuchtmethoden Dieser Artikel ist leider nicht in deutscher Sprache verfügbar. 
2 Methods of Plant Breeding
2.1 Conventional Methods
Plant breeding is defined as identifying and selecting desirable traits
in plants and combining these into one individual plant. Since 1900,
Mendel's laws of genetics provided the scientific basis for plant
breeding. As all traits of a plant are controlled by genes located on
chromosomes, conventional plant breeding can be considered as
the manipulation of the combination of chromosomes. In general,
there are three main procedures to manipulate plant chromosome
combination. First, plants of a given population which show desired
traits can be selected and used for further breeding and cultivation,
a process called (pure line-) selection. Second, desired traits found
in different plant lines can be combined together to obtain plants
which exhibit both traits simultaneously, a method termed
hybridization. Heterosis, a phenomenon of increased vigor, is
obtained by hybridization of inbred lines. Third, polyploidy (increased
number of chromosome sets) can contribute to crop improvement.
Finally, new genetic variability can be introduced through spontaneous
or artificially induced mutations.[2]
- Selection
Selection is the most ancient and basic procedure in plant breeding.
It generally involves three distinct steps. First, a large number of
selections are made from the genetically variable original population.
Second, progeny rows are grown from the individual plant selections
for observational purposes. After obvious elimination, the selections
are grown over several years to permit observations of performance
under different environmental conditions for making further eliminations.
Finally, the selected and inbred lines are compared to existing
commercial varieties in their yielding performance and other aspects
of agronomic importance.y
- Hybridization
The most frequently employed plant breeding technique is
hybridization. The aim of hybridization is to bring together desired
traits found in different plant lines into one plant line via cross-
pollination. The first step is to generate homozygous inbred lines.
This is normally done by using self-pollinating plants where pollen
from male flowers pollinates female flowers from the same plants.
Once a pure line is generated, it is outcrossed, i. e. combined with
another inbred line. Then the resulting progeny is selected for
combination of the desired traits. If a trait from a wild relative of a
crop species, e.g. resistance against a disease, is to be brought into
the genome of the crop, a large quantity of undesired traits (like low
yield, bad taste, low nutritional value) are transferred to the crop as
well. These unfavorable traits must be removed by time-consuming
back-crossing, i. e. repeated crossing with the crop parent. There are
two types of hybrid plants: interspecific and intergeneric hybrids.
Beyond this biological boundary, hybridization cannot be
accomplished due to sexual incompatibility, which limits the
possibilities of introducing desired traits into crop Plants.
Heterosis is an effect which is achieved by crossing highly inbred lines
of crop plants. Inbreeding of most crops leads to a strong reduction
of vigor and size in the first generations. After six or seven generations,
no further reduction in vigor or size is found. When such highly inbred
plants are crossed with other inbred varieties, very vigorous, large sized,
large-fruited plants may result. The term "heterosis" is used to describe
the phenomenon of hybrid vigor. The most notable and successful
hybrid plant ever produced is the hybrid maize. By 1919, the first
commercial hybrid maize was available in the United States. Two
decades later, nearly all maize was hybrid, as it is today, although
the farmers must buy new hybrid seed every year, because the
heterosis effect is lost in the first generation after hybridization of the
inbred parental lines.
- Polyploidy
Most plants are diploid. Plants with three or more complete sets of
chromosomes are common and are referred to as polyploids. The
increase of chromosomes sets per cell can be artificially induced by
applying the chemical colchicine, which leads to a doubling of the
chromosome number. Generally, the main effect of polyploidy is
increase in size and genetic variability. On the other hand, polyploid
plants often have a lower fertility and grow more slowly.
- Induced mutation
Instead of relying only on the introduction of genetic variability from
the wild species gene pool or from other cultivars, an alternative is
the introduction of mutations induced by chemicals or radiation. The
mutants obtained are tested and further selected for desired traits.
The site of the mutation cannot be controlled when chemicals or
radiation are used as agents of mutagenesis. Because the great
majority of mutants carry undesirable traits, this method has not
been widely used in breeding programs.[2]
Success in using conventional plant breeding principles and agricultural
techniques reached its peak when high-yielding wheat and rice lines
were cultivated in the 1960s. The doubling and tripling of productivity
of these important crops in Asia signaled a agricultural revolution in
the developing countries. This breakthrough in food production was
termed the "Green Revolution" to describe the social, economic, and
nutritional impact of the new high-yielding wheat and rice strains.
Norman Borlaug was awarded the Noble Price in 1970 for his
contribution in breeding new high-yielding strains of cereals. However,
these strains were highly dependent on fertilizers, irrigation and
agrochemicals and required energy-intensive investments. This led
to degradation and loss of soils and other severe environmental
problems all over the world. For these reasons, the "Green Revolution"
has been both praised and damned.[2]
2.2 Biotechnological Methods
Biotechnology is the discipline which deals with the use of living
organisms or their products. In this wide sense, also traditional
agriculture may be seen as a form of biotechnology. The European
Federation of Biotechnology defines biotechnology as "the integrated
use of biochemistry, microbiology and engineering sciences in order
to achieve technological (industrial) application of the capability of
microorganisms, cultured tissue cells and parts thereof". In recent
years, biotechnology has developed rapidly as a practical means for
accelerating success in plant breeding and improving economically
important crops. The most important methods used to achieve these
goals are described below. The techniques of genetic engineering,
which are a part of biotechnology, will be discussed in more detail in
the next chapter.
- In Vitro Cultivation of Plant Cells and Regeneration of Plants from
Cultured Cells Certain isolated somatic plant cells can be cultured in
vitro (in the test tube) and are capable of proliferation and organization
into tissues and eventually into complete plants. The process of
regenerating whole plants out of plant cells is called in vitro regeneration.
The three factors affecting plant regeneration are genotype, explant
source, and culture conditions, including culture medium and
environment. Different mixtures of plant hormones and other
compounds in varying concentrations are used to achieve regeneration
of plants from cultured cells and tissues. As the plant hormonal
mechanisms are not yet understood completely, the development
of in vitro cultivation and regeneration systems is still largely based
on empirically testing variations of the three above mentioned
factors. [3]
- In Vitro Selection and Somaclonal Variation
Plants regenerated from cell cultures may exhibit phenotypes differing
from their parent plants, sometimes at quite high frequencies. If these
are heritable and affecting desirable agronomic traits, such "somaclonal
variation" can be incorporated into breeding programs. However,
finding of specific valuable traits by this method is largely left to
chance and hence inefficient. Rather than relying on this undirected
process, selection in vitro targets specific traits by subjecting large
populations of cultured cells to the action of a selective agent in the
Petri dish. For purposes of disease resistance, this selection can be
provided by pathogens, or isolated pathotoxins that are known to
have a role in pathogenesis. The selection will only allow those cells
to survive and proliferate that are resistant to the challenge. Selection
of cells also plays an important role in genetic engineering, where
special marker genes are used to select for transgenic cells (see below).
[4]
- Somatic Hybrid Plants
Somatic hybrid plants are plants derived from the fusion of somatic
cells. Cell fusion was developed after the successful culture of a large
number of plant cells stripped of their cell walls. The resulting cells
without walls are referred to as protoplasts. Since also protoplasts
from phylogenetically unrelated species can be fused, attempts have
been made to overcome sexual incompatibility using protoplast fusion.
In most cases, these attempts failed because growth and division of
the fused cells did not take place when only distantly related cells were
fused. Successful fusions between sexually incompatible petunia
species and between potatoes and tomatoes did not lead to
economically interesting products, but important contributions to the
understanding of cell wall regeneration and other mechanisms were
achieved. [2]
2.3 Genetic Engineering
Genetic engineering is a term used for the directed manipulation of
genes, i. e. the transfer of genes between organisms or changes in
the sequence of a gene. Closely related to this field are methods
which use genes or specific sequences for the identification of traits
and other analytical purposes. In plant breeding, the most important
and already widely used method of this kind is Restriction Fragment
Length Polymorphism (RFLP). The basics of this technique are
described below. The principles of plant genetic engineering will be
described in the next chapter. For those who are not familiar with
the principles of genetics, the glossary at the end of this report
explains the most important terms.
2.3.1 Restriction Fragment Length Polymorphism (RFLP)
The techniques of traditional breeding are very time-consuming. By making
crosses, also a large number of undesired genes is introduced into
the genome of the plant. The undesired genes have to be "sorted out"
by back-crossing. The use of Restriction Fragment Length Polymorphism
greatly facilitates conventional plant breeding, because one can
progress through a breeding program much faster, with smaller
populations and without relying entirely on testing for the desired
phenotype.
RFLP makes use of restriction endonucleases. These are enzymes which
recognize and cut specific nucleotide sequences in DNA. For example,
the sequence GAATTC is cut by the endonuclease EcoRl. After treatment
of a plant genome which restriction endonucleases, the plant DNA is
cut into pieces of different length, depending on the number of
recognition sites on the DNA. These fragments can be separated
according to their size by using gel electrophoresis and are made
visible as bands on the gel by hybridizing the plant DNA fragments
with radiolabeled or fluorescent DNA probes. As two genomes are not
identical even within a given species due to mutations, the number of
restriction sites and therefore the length and numbers of DNA fragments
differ, resulting in a different banding pattern on the electrophoresis
gel. This variability has been termed restriction fragment length
polymorphism (RFLP). The closer two organisms are related, the more
the pattern of bands overlap. If a restriction site lies close to or
even within an important gene, the existence of a particular band
correlates with the particular trait of a plant, e.g. disease
resistance. By looking at the banding pattern, breeders can identify
individuals which have inherited resistance genes, and resistant
plants can be selected for further breeding. The use of this technique
will not only accelerate progress in plant breeding considerably, but
will also facilitate the identification of resistance genes, thereby
opening new possibilities in plant breeding (compare also chapter
3.1.3.1).[4]
2.3.2 Gene Transfer
In conventional breeding, the pool of available genes and the traits they
code for is limited due to sexual incompatibility to other lines of
the crop in question and to their wild relatives. This restriction
can be overcome by using the methods of genetic engineering, which
in principle allow introducing valuable traits coded for by specific
genes of any organism (other plants, bacteria, fungi, animals, viruses)
into the genome of any plant. The first gene transfer experiments
with plants took place in the early 1980s. Normally, transgenes are
inserted into the nuclear genome of a plant cell. Recently it has
become possible to introduce genes into the genome of chloroplasts
and other plastids (small organelles of plant cells which possess a
separate genome). The advantages of this technique are discussed in
chapter 3.1.1.1.
Transgenic plants have been obtained using Agrobacterium-mediated DNA-transfer
and direct DNA-transfer, the latter including methods such as
particle bombardment, electroporation and polyethylenglycol
permeabilisation. The majority of plants have been transformed using
Agrobacterium mediated transformation.[5]
2.3.2.1 Agrobacterium-mediated Gene Transfer
The Agrobacterium-mediated technique involves the natural gene transfer
system resident in the bacterial plant pathogens of the genus
Agrobacterium. In nature, Agrobacterium tumefaciens and Agrobacterium
rhizogenes are the causative agents of the crown gall and the hairy
root diseases, respectively. The utility of Agrobacterium as a gene
transfer system was first recognized when it was demonstrated that
these plant diseases were actually produced as a result of the transfer
and integration of genes from the bacteria into the genome of the plant.
Both Agrobacterium species carry a large plasmid (small circular DNA
molecule) called Ti in A. tumefaciens and Ri in A. rhizogenes. A segment
of this plasmid, designated T-(for transfer) DNA, is transmitted by
this organism into individual plant cells, usually within wounded
tissue (see figure 2.1). The T-DNA segment penetrates the plant cell
nucleus and integrates randomly into the genome where it is stably
incorporated and inherited like any other plant gene in a predictable,
dominant Mendelian fashion. Expression of the natural genes on the
T-DNA results in the synthesis of gene products that direct the
observed morphological changes, i. e. tumor or hairy root
formation.
In genetic engineering, the tumor inducing genes within the T-DNA which
cause the plant disease are removed and replaced by foreign genes.
These genes are then stably integrated into the genome of the plant
after infection with the altered strain of Agrobacterium, just like
the natural T-DNA. Because all tumor-inducing genes are removed, the
gene transfer does not induce any disease symptoms. The most important
steps in Agrobacterium-mediated gene transfer are shown in figure
2.1.This reliable method of gene transfer is well suited for plants
which are susceptible to infection by Agrobacterium. Unfortunately,
many species, especially economically important legumes and
monocotyledons such as cereals, do not respond positively to
Agrobacterium-mediated transformation. For these plants, the following
methods of direct DNA uptake must be applied.[5]
2.3.2.2 Particle Bombardment
This method, also referred to as biolistic transformation (from biological
ballistics) involves coating biologically active DNA onto small
tungsten or gold particles (1-5 ?m in diameter) and accelerating them
into plant tissue at high velocity. The particles penetrate the plant
cell wall and lodge themselves within the cell where the DNA is
liberated resulting in transformation of the individual plant cell in
an explant. This technique is generally less efficient than
Agrobacterium-mediated transformation, but has nevertheless been
particularly useful in several plant species, most notably in cereal
crops. The introduction of DNA into organized, morphogenic tissues
such as seeds, embryos or meristems has enabled the successful
transformation and regeneration of rice, wheat, soybean and maize,
thus demonstrating the enormous potential of this method .[5]
2.3.2.3 Electroporation and Direct DNA Entry into Protoplasts
Electroporation is a process whereby very short pulses of electricity are
used to reversibly permeabilize lipid bilayers of plant cell membranes.
The electrical discharge enables the diffusion of macromolecules such
as DNA through an otherwise impermable plasma membrane. Because the
plant cell wall will not allow the efficient diffusion of many transgene
constructs, protoplasts (cells without cell walls) must be prepared.
This requirement presents a major obstacle for many applications as
protocols making possible the regeneration of protoplasts into complete
plants do not exist for many species.
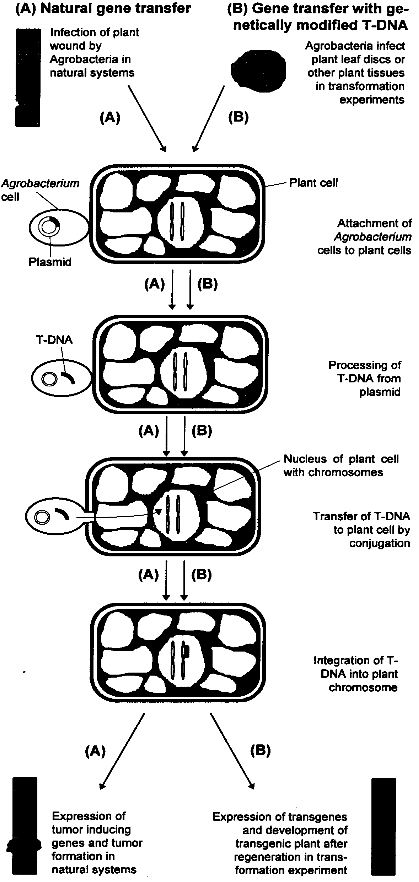
Fig. 2.1: Gene transfer from Agrobacterium tumefaciens to a plant cell.
In nature (route A), the transfer DNA (T-DNA) contains tumor inncluding
genes which lead to crown gall disease. In plant genetic engineering
(route B), the tumor including genes are removed and replaced by other
genes, e.g. genes which confer insect resistance. In nature as well as
in genetic engineering, the agrobacteria attach to a plant cell. Then,
the T-DNA is cut out of a plasmid (a small circular DNA-molecule) and is
transfered to the plant cell. The T-DNA migrates to the nucleus of the
plant cell and becomes incorporated into a plant chromosome. In nature
(route A), the tumor inducing genes cause uncontrolled (tumor-like)
growth at the site of infection after expression of the T-DNA. In
genetic engineering (route B, tumor inducing genes removed by other
genes), transgenic cells are selected and regenerated to whole plants.
All cells of the regenerated plant now contain the transgene. Adapted
from [6]
DNA uptake by plant protoplasts can also be stimulated by phosphate or
calcium/polyethylene glycol (PEG) coprecipitation. However, these
methods all suffer from the drawback that they use protoplasts as the
recipient host which often cannot be regenerated into whole plants.
[5]
2.3.3 Transgene Expression
In most cases, the introduction of a gene into the plant genome will only
have an effect on the plant if the transgene is expressed, i. e.
transcribed into mRNA and translated into a protein. A promoter is a
sequence of nucleic acids where the RNA polymerase (a complex enzyme
synthesizing the mRNA transcript) attaches to the DNA template. The
nature of the promoter defines (together with other expression-regulating
elements), under which conditions and with which intensity a gene
will be transcribed. The promoter of the 35S gene of cauliflower mosaic
virus is used very frequently in plant genetic engineering. This
promoter confers high-level expression of exogenous genes in most
cell types from virtually all species tested. As it is often
advantageous to express a transgene only in certain tissues or
quantities or at certain times, a number of other promoters are
available, e.g. promoters inducing gene expression after wounding or
during fruit ripening only.
Methods of gene transfer currently employed result in the random integration
of foreign DNA throughout the genome of recipient cells. The site of
insertion may have a strong influence on the expression levels of the
exogenous gene, resulting in different expression levels of an
introduced gene, even if the same promoter/gene construct was used.
The exact mechanism of this phenomenon are not yet fully understood.
[5]
2.3.4 Selection and Plant Regeneration
In a transformation experiment, the proportion of transformed cells is
usually small compared to the number of cells which remain unaltered.
In order to select only cells which have actually incorporated the
new genes, the genes coding for the desired trait are fused to a gene
which allows selection of transformed cells, so-called marker genes.
The expression of the marker gene enables the transgenic cells to
grow in presence of a selective agent, usually an antibiotic or a
herbicide, while cells without the marker gene die. One of the most
commonly used marker genes is the bacterial aminoglycoside-3'
phosphotransferase gene (APH(3')II), also referred to as neomycin
phosphotransferase 11 (NPTII). This gene codes for an enzyme which
inactivates the antibiotics kanamycin, neomycin and G418 through
phosphorylation. In addition to NPTII, a number of other antibiotic
resistance genes have been used as selective markers, e.g. hygromycin
phosphotransferase gene conferring resistance to hygromycin.
Another group of selective markers are herbicide tolerance genes. Herbicide
tolerance has been obtained through the incorporation and expression
of a gene which either detoxifies the herbicide in a similar manner
as the antibiotic resistance gene products or a gene that expresses
a product which acts like the herbicide target but is not affected
by the herbicide. Herbicide tolerance may not only serve as a trait
useful for selection in the development of transgenic plants, but
also has some commercial interest. Herbicide tolerance transgenic
plants are therefore among the first crops approaching market
introduction.
Transformation of plant protoplasts, cells and tissues is usually only
useful if the they can be regenerated into whole plants. The rates
of regeneration vary greatly not only among different species, but
also between cultivars of the same species. As mentioned in capter
2.2, in many cases regeneration of whole plants from cells is not
possible or very difficult. Besides the ability to introduce a gene
into the genome of a plant species, regeneration of intact, fertile
plants out of transformed cells or tissues is the most limiting step
in developing transgenic plants.[5]
|